Precision landing capabilities to deliver spacecraft to safe landing locations in close proximity of targeted exploration sites is a high priority for human and robotic missions to the Moon, Mars, and elsewhere in the solar system. While significant efforts have gone into the development of precise navigation capabilities, challenges remain with the detection of safe landing sites, especially sites within permanently shadowed regions or sites that are hazardous for safe and accurate landing near lunar dawn where the low-lighting and long shadows present technical challenges for current landing systems. Risk reduction for such missions will require further advancement of sensing systems used for the onboard generation of high-resolution maps for hazard detection. In addition, current capabilities do not address the size, weight, power, and cost (SWaP-C) requirements that will help enable upcoming missions. Advancements are needed to make these sensors smaller, lighter, more cost effective, and straightforward to integrate with a variety of lander vehicles.
Why is it so important to develop the capability to land in the dark on the Moon? This visualization produced by NASA Goddard gives the viewer a good sense of just how dynamic the lighting and thermal environments are in the resource-rich, permanently shadowed regions (PSR’s) near the Moon’s South Pole.
Video credit: NASA's Goddard Space Flight Center
NASA is interested in advancing spacecraft landing capabilities that include sensing of planetary surface terrains in the dark from an altitude of 250 meters or higher. The intent of this competition is to identify sensor systems (e.g., radar, LIDAR, optical) capable of generating three dimensional maps during rocket-powered landing maneuvers with enough accuracy and resolution to be used for hazard detection and landing site selection. Successful sensor systems will drive down the size, mass, power, and cost beyond the current state of the art. Winners will also have the opportunity to compete for additional funding and flight testing through the Payout Build Phase and Performance Incentive Phase.
To be competitive, your application should describe how your technology will meet these technical guidelines:
- Able to be demonstrated on a rocket-powered suborbital vehicle.
- Able to perform autonomous terrain mapping of a 250-meter diameter region within a lunar surface test field, in the dark, without a priori knowledge of the terrain features or their locations (see further details on lunar surface test field in the Performance Incentive Phase section below)
- Able to generate the map in an onboard-reference-frame defined at a single time just before data collection from an altitude of at least 250 meters. The maps generated during the flight test will be compared to a high resolution “truth map” previously generated through a terrestrial georeferencing LIDAR service and should achieve:
- ~~~Spatial resolution:10 cm ground sample distance (GSD) or better
- ~~~Elevation accuracy: less than 5 cm (1-sigma) elevation error
- ~~~Lateral accuracy: features correctly localized within 10-cm GSD (or better)
- The payload must not exceed the following requirements:
- ~~~Maximum Mass of Sensor: 20 kg
- ~~~Maximum Mass of Total Payload: 50 kg
- ~~~Note: Teams will need to provide power for their payload during the flight test
- ~~~Maximum Volume: 355 mm tall x 724 mm diameter
- ~~~An arm extending to the side for a downward view is allowed. The arm should not extend beyond 300 mm from the vehicle side and shall not have a moment greater than 30 N·m (10 kg max at 300 mm).
- The payload must be able to be activated remotely during flight testing. Note: The intention is that payloads will be recovered at the end of each flight test.
For this challenge teams are not required to build or test spaceflight qualified sensor systems. However, less massive and more cost-effective space-qualified sensors for hazard detection are the eventual end-goal for NASA. Applicants should describe the path leading to flight hardware development for their sensor system technologies. Elements to consider include materials selection, mass, volume, power, and computing capability to enable the system to operate in the thermally challenging, vacuum, high-radiation lunar environment.
PayLoad Build Phase
In addition to the $200,000 prize awarded at selection, Winners will have the opportunity to compete for an award of $200,000 each during Payload Build Round 1. Winners will participate in a Payload Build kick-off call to present their plans for the payload development and progress to date. Field Judges will conduct an on-site visit in October 2022 to score the progress each Winner has made to determine if they qualify for the Round 1 award.
Upon successful completion of Payload Build Round 1, Winners will have the opportunity to compete for an additional award of $100,000 each in Payload Build Round 2. Winners will participate in a conference call to present their progress to date on their plans for the payload development. Field Judges will conduct an on-site visit in March 2023 to score the progress each Winner has made to determine if they qualify for the Round 2 award.
Performance Incentive Phase
Upon successful completion of Payload Build Round 2, NASA intends to provide each Winner a suborbital flight test at no additional cost and the opportunity to compete for an additional award of up to $150,000 each based on the performance of their system during the flight test.
To win an award during the flight test in the Performance Incentive Phase, a Winner must generate a map that meets the minimum map quality requirements at an altitude of 250 m or greater and within the timeline of the vehicle flight profile. Prize amounts will be based on the altitude at which the map is electronically transmitted to the lander vehicle, which will time stamp the map for post-flight assessment of the vehicle altitude corresponding to the map transmission.
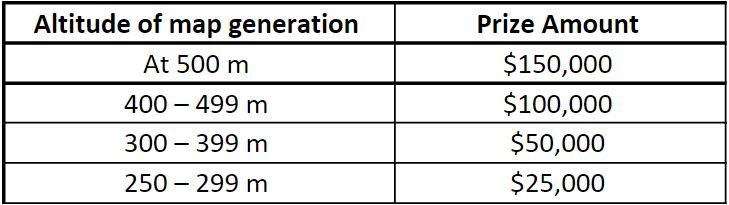
For this challenge, NASA intends to use a rocket-powered suborbital vehicle with a flight profile that includes a controlled descent with a controlled vertical landing and anticipates contracting with Masten Space Systems.
NASA anticipates the following for the flight tests:
- Test will be performed at Masten Space Systems’ test site located at the Mojave Air and Space Port. Terrain field hazards (e.g., rocks, slopes, craters) will be implemented in a lunar surface test field by NASA and will be unknown to the Winners.
- Tests will be conducted at night, during a new moon or prior to moonrise.
- ~~~Free of any clouds below 10,000 ft above sea level (ASL)
- ~~~Free of wind exceeding 15 knots (sustained) with the vehicle take-off position downwind to avoid dust encroachment into the lunar surface test field
- During the flight, NASA anticipates the vehicle will:
- ~~~Take off from a concrete pad adjacent the lunar surface test field to an altitude of 500 meters above ground level (AGL)
- ~~~Translate horizontally to optimize the sensor’s view of the lunar surface test field
- ~~~Remain stationary at 500 meters for 2 seconds
- ~~~Descend straight down to 250 meters AGL
- ~~~Descent maneuver will be at a rate of 25 meters per second, matching the expected descent rate for most lunar landers
- ~~~Remain stationary at 250 meters for 2 seconds
- ~~~Translate to a landing pad outside of the lunar surface test field
- The lunar surface test field will consist of:
- ~~~An area of equal to or greater than 100 meters in diameter
- ~~~A variety of hazards, to include features such as rocks (~10 cm), craters (>1 m diameter), steep-walled rilles, and various slopes of up to 30 degrees
- ~~~A regolith simulant with high reflectivity
- ~~~Lighting at low angles
- Flight provider will provide an interface control document that outlines data communication with the lander as well as information about physical mounting options
- Once the suborbital flight test has been completed, each Winner will submit a final report, discussing flight test results and plans for the future of the technology.
Challenge Context
Near the lunar poles, low-angle lighting and steep topography create permanently shadowed regions (PSRs). Lunar mission architecture planners must contend with rugged terrain, crowded with hazards, in places that light never reaches. However, these challenging lighting conditions and hazardous terrain are what concentrate and hold the Moon’s water ice. Water ice is one of the Moon’s most valuable resources and thus a target of great interest. As such, NASA is supporting the advancement of precision landing and hazard detection capabilities to deliver spacecraft to safe landing locations within reach of PSRs. These systems must be smaller and less massive than what is currently space qualified for such missions.
As an example, the development of miniaturized spacecraft (CubeSats and others) has revolutionized approaches to orbital missions. Driving down the size and mass of these small spacecraft has provided access to space to entities and individuals that would have otherwise been unable to participate in such missions. To bring this type of revolution to the exploration of planetary surfaces, the size and mass must be reduced for the sensing systems that will be required to detect hazards and produce high-resolution maps, which will also eventually calculate a solution that not only considers the safety of a landing site but also optimizes the landing location based on the mission objectives (e.g., expediency of reaching mission targets.)